Quantum Sensors: Atomic Details, Monumental Impact
Quantum sensors detect subatomic and atomic shifts, being innovated on to image and detect certain intracellular qualities and processes that provides a promising future in the medical field.
Reading Time: 5 minutes
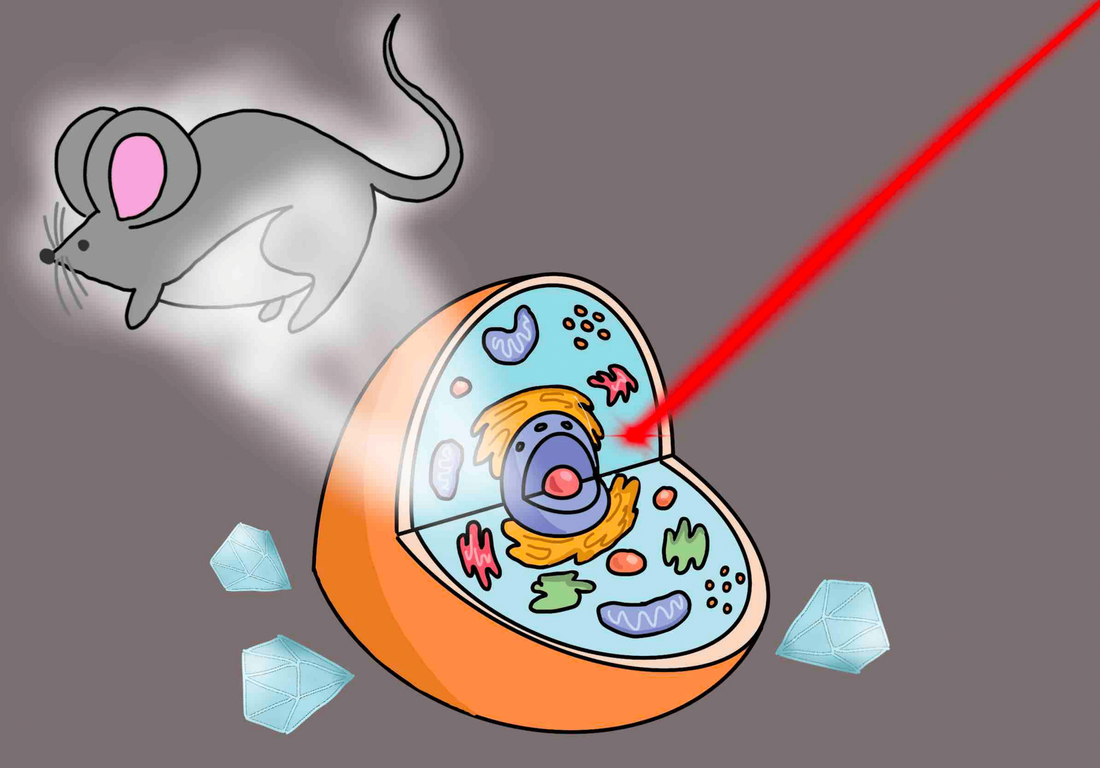
In the macroscopic world, objects can adequately be described as particles; yet quantum mechanics introduces that at the atomic level, small objects can act as both particles and waves. This duality introduces unique characteristics that can be manipulated into creating cutting-edge technology. This includes quantum sensors that measure infinitesimally small changes with great precision, something with widespread applications beyond quantum physics.
Quantum sensors detect small changes in external factors. These small changes are detected by exploiting the fragility of quantum states, a description of the physical world in quantum mechanics. In these systems, particles are able to maintain quantum superposition, or the ability to hold multiple values, for periods of time known as their coherence times. During this time, these particles have properties that coexist simultaneously as a distribution of all possibilities. These distributions change when external factors such as time, temperature, pressure, frequency, acceleration, and magnetic and electric fields change. The change in this distribution can be observed with great precision by quantum sensors, allowing for accurate information about these changes.
One of the growing applications of quantum sensors is in the field of biomedicine. In this field, two forms of quantum sensors are prominent: optically pumped atomic magnetometers (OPM) and Nitrogen-vacancy (NV) centers. Both utilize spin, which is a particle’s angular momentum, and spin states, or the configuration of spins, for quantum sensing.
In OPMs, a light source optically pumps atoms into their vapor form, where atoms exist as unbonded, individual units, aligning their electron spins and consequently making the vapor sensitive to magnetic fields. Thus, when an external field is present, the spin state shifts, causing polarization or absorption of the light source to change when passing through the vapor. When the light changes, its difference is proportional to the strength and direction of the magnetic field—helping detect and identify the field present.
Imaging is a main component of the field of radiology, which utilizes magnets and radio waves in magnetic resonance imaging to view structures in the body. With OPMs, another form of brain imaging has been developed. Magnetoencephalography (MEG) involves measuring small magnetic fields formed from the electrical currents of the brain, allowing for sub-millisecond detection of neuron activity to be mapped. MEGs are generally a stationary large structure with a seat and a large roof that contains the sensors that surround the head. Initially, the main sensors used in MEG were superconducting quantum interference devices (SQUIDs), which were effective at detection yet prone to other problems.
The SQUIDs in MEG devices were very bulky and restricted major head movements due to the need for cryogenic cooling, where liquid helium was required to allow the sensors to function. Liquid helium has an extremely low boiling point, allowing it to maintain the proper electrical conductivity and strength for the machine. Superconductivity relies on the low temperature which enables substances to conduct currents of electricity without losing too much energy from the transfer. However, a new form of MEG technology utilizing OPMs has been discovered to have a relatively similar detection sensitivity to SQUID systems but without requiring the cooling process.
This allows for more mobility, customization for patients, and efficiency of data retrieval. OPMs also allow the device to be closer to the scalp of patients, enabling more detailed data compared to the bulky structure of SQUID based MEGs. This is especially critical in the usage of MEGs because the lighter structure would allow for devices that would fit the heads of child patients. Since OPMs rely on light while SQUIDs rely on cryogenics to achieve superconductivity in sensors, this form of quantum sensor has paved a breakthrough in the way pediatric neuroscience and movement-based neurology can be studied.
The NV center is a point defect in diamond, a carbon lattice, where one carbon atom is replaced by a nitrogen atom that is adjacent to a missing atom in the structure. The NV center has spin states that are highly sensitive to changes in magnetic fields. When NV centers are exposed to a magnetic field, nuclear spin states oscillate at a frequency dependent on the field’s strength and direction using a technique known as nuclear magnetic resonance spectroscopy, which allows for small changes in magnetic fields to be determined. The NV centers are then optically pumped and the emitted light changes based on spin. Then, NV centers are optically pumped with laser light to analyze factors of photoluminescence, which change based on spin states, to determine information about the magnetic field.
One particularly notable discovery was from the creation of nanodiamonds using negatively charged NV centers. Nanodiamonds range from four to five nanometers in size but are very physically and chemically strong crystalline carbon structures. They can conjugate easily with molecules inside and outside of the formation by bonding more efficiently. Their large surface area, curved surface of unstable electrons from the interchangeability of the bonds shifting, and ability to be stable in water allows different external molecules to access the outer bonds of the nanodiamond structure.
These qualities enable the nanodiamonds to bind with water-soluble drugs, allowing for the molecules inside to be easily traced. The fluorescent abilities of the nanodiamonds let researchers view the movement of the drugs through cells or tissues easily by glowing with enough specificity to determine the route of the treatment. The NV centers are important for the fluorescence of certain nanodiamonds as they are the defects created during centrifugation creating colored centers that can’t be bleached away like organic dyes, such as proteins like GFP.
Compared to protein dyes, nanodiamonds are smaller and can be utilized in a multitude of organisms instead of just bacteria or certain cells which need to first encode the genetic material needed to produce more fluorescent protein. On top of that, the nanodiamonds may even contain spin properties that allow them to react in limited magnetic fields, establishing a way to use them for sensors. Nanodiamonds provide a great alternative to organic dyes because they offer a much better view of complex structures, which vary from the smallest cell to whole body scans, while easily entering and exiting cells without complications.
With the rapid innovation of new technology, the collaboration between physics and biology will bring quantum sensors into more active use. Connecting the atomic world and the physical world seems incomprehensible at first glance. However, as scientists learn more about our smallest levels of classification, from studying the atoms in a molecule to the molecules in a cell, the applications of quantum mechanics for medicine will skyrocket. From the present onwards, we will continue to bridge the macroscopic and microscopic world to understand the mysteries of imaging.